The left figure above shows
currents measured during the 3rd stroke of a 9
stroke flash.
The current measured at point A is the largest
probably because it was closest to the current
injection. Actually the peak current
measured at point A is larger than the peak value
of the injected current. This could be
because there was coupling into a loop formed by
the LPS (the roof wire, the 2 down conductors, and
the ground form a large loop) and also because of
coupling into the measuring system which might not
have been shielded properly.
The current waveforms measured at points A, B, and
C are narrower than the injected current
waveform. It appears that mainly the high
frequencies in the injected current waveform
travel to the local ground, the lower frequencies
travel through point D and into the house wiring
(note that the current flowing through pt. D and
into the house wiring, reaches a peak value of
just under 3 kA). The grounding rods act
more like a capacitive impedance to ground than a
resistive impedance.
The waveforms at points D and G, shown in
the right figure above are from stroke 7 in the
flash (stroke 7 had the lowest injected peak
current value) . The two waveforms are very
similar, indicating that the current flowing
through point D eventually flows to ground through
point G at the location 50 m from the
house. The arcing shown on the
figure was current from a different experiment
sparking across to the grounding rod at point
G.
Currents measured at point D (i.e.
currents flowing into the house wiring) ranged
from about 1 to about 3 kA for the 9 stroke
flash. Overall currents measured at pt. D
ranged from 16% to 28% of the peak value of the
injected current. This was considerably less
than was observed in the earlier (1997) experiment
and more in line with expectations.
I was surprised to learn that currents of this
amplitude could potentially enter a home on the
wiring in the event of a direct strike. And
this is a house with a LPS, which would seem like
a best-case scenario. However, there is no
mention of a circuit breaker or fuses at the point
where the electrical service entered the test
house. Presumably if lightning were to
strike the LPS of a real house, the fuses and/or
circuit breaker would prevent currents of this
intensity from flowing into a house.
In a home the
first line of defense could be surge protection
outside the home at the circuit breaker box where
electrical service arrives or where the cable TV
or phone line signals connect to a house.
Once in the home plugging sensitive electronic
equipment into a surge protector would offer
additional protection against transients still on
the power line. Electronics equipment itself
will often have surge protection installed on
circuit boards inside a device.
What might the transient protection look
like. In the most general terms it will
probably consist of a blocking impedance followed
by a shunt impedance.
The blocking
impedance should present a high impedance to the
transient signal to prevent it from reaching the
electronics. The shunt has a low impedance
and will divert the transient signal to ground.
For the signal of interest, Z1 should appear
as a low impedance. You might use an
inductor if the incoming signal is low frequency
(60 Hz power for example) and you want to block
high frequency transients. If the incoming
signal is high frequency, a capacitor would block
low frequency transients.
The shunt resistance should appear as a high
impedance for the signal of interest, a capacitor
if the incoming signal is low frequency.
I have been taught that it is a good idea to
provide an alternate path to ground for an
incoming signal that encounters a blocking
impedance (though the blocking impedance may also
cause the transient signal to be reflected rather
than diverted). I.e. something like the
following:
The first component is often a gas discharge tube.
A gas discharge
tube or "spark gap" is an example of a "crowbar"
device. It creates a short circuit (like
putting
a crowbar across the signal leads) once
it exceeds a certain voltage threshold. The
figure below (from Uman's lightning protection
book) shows the operating characteristics of a
typical gas discharge tube.
The spark gap
depicted here quickly becomes conducting once a
voltage threshold of about 600 volts is
crossed. Spark gaps can carry large
currents and are bipolar. They turn on
relatively slowly however (~1 μs). Note
that once created the arc discharge can be
maintained even at low current levels. A
device like this is sometimes difficult to "turn
off."
The role of the gas discharge tube is not to
carry or divert all of the transient signal to
ground. Rather the transient signal is
reflected back in the direction it came
from. A short discussion of reflection at
the end of a short circuited line and a line
with infinite impedance (open circuit) has been
added to the end of today's notes.
MOV in the figure stands for metal oxide
varistor. A varistor is a
voltage-controlled resistor. The operating
characteristics are shown below (again from
Uman's book). Varistors are clamping
devices which means they hold or limit the
voltage to a particular value.
In the figure above, the varistor becomes
active when the voltage across it reaches
perhaps 180 volts (prior to that it has a
large impedance). The voltage is then
held at about that value until the current
through the varistor reaches perhaps 100 A
(currents higher than that would presumably
destroy the device). A 200 v clamping
voltage would be appropriate for a 110 volt
AC power line. MOVs turn on very
quickly (nanoseconds) and are bipolar.
They do however have a relatively high
capacitance and are not able to divert
overvoltages for a sustained period of time.
The MOV could be followed by another
shunt impedance such as a zener diode which
would clamp the incoming signal to an even
lower voltage. This is sketched
below. Each device adds some
additional attenuation of the transient
signal.
The figure below
contrasts the operation of an ordinary
diode and a zener diode.
The left figure
shows a conventional diode.
The diode begins to conduct when the
forward bias voltage reaches about
0.65 volts. Very little
current flows through the diode when
back biased. The zener diode
at right operates in the same way
when forward biased.
When back biased the diode doesn't
conduct much current until the
voltage reaches a breakdown or zener
voltage (Vz). Then
voltage will be maintained at Vz
over a wide range of currents.
In addition to use as a surge
protective device, zener diodes are
also used as voltage
regulators. Note that a zener
diode is not a bipolar device, it
offers surge protection against only
one polarity of overvoltage.
Many of the devices we have been
discussing can be found inside a
commercial surge protector (in this
case an Inmac 8215 Surge Protector)
A gas
discharge tube can be seen near
the center left edge of the
printed circuit board (white
ceramic case). A total of
6 MOVs can be seen. They
are green, circular, and might
otherwise be mistaken for
capacitors. There is room
for two inductors on the circuit
board (the two circles printed
on the right side of the circuit
board). The inductors are
apparently not included in this
model.
Finally a few notes from the
chapter on medical issues and personal
lightning safety in Uman's lightning
protection book.
Despite a large increases in total
population, the number of people
killed by lightning every year in the
United States has dropped by an order
of magnitude in the last century or so
(from more than 400 per year in the
early 1900s to about 30 per year at
the present time). The same is
true in many other developed countries
in the world. The most recent
estimates that I could find are
summarized below (adapted from Holle
(2015)).
This decrease has largely been due
to movement of people from rural to
urban locations and into safer homes,
workplaces, and schools. An
additional reason for the decrease is
travel is now almost always inside a
vehicle with a metal body (that acts
as a Faraday cage). There
are about 10 lightning injuries
(requiring medical attention) per
lightning death.
Source
of the image above.
The figure of 6 deaths per million is
thought to apply still in regions
where large portions of the population
live in rural areas with an
agriculture based economy.
About 24,000 lightning deaths
occur per year around the globe
(figure above adapted from Holle
(2015))
A breakdown of lightning deaths by
activity (from Jensenius (2016))
The primary causes of death from
lightning are cardiopulmonary arrest
and damage to the central nervous
system. I had always thought
that someone struck by lightning would
be seriously burned (internally and
externally). This is apparently
not the case, the duration of the
lightning current is too short.
Sometimes a person's clothing catches
on fire, however, and that can cause
serious burns.
A lightning strike can cause eye
damage and hearing loss (one or both
eardrums is(are) often
ruptured). Psychological effects
(anxiety, fatigue, chronic headaches
or other pain, personality changes and
depression) are apparently a
significant and long-lasting result of
a lightning strike.
Telephone injury is the most common
type of indoors injury associated with
lightning.
I would encourage you to read "Updated
Recommendations for Lightning Safety
- 1998" by R.L. Holle, R.E.
Lopez and C. Zimmerman if you ever
find yourself in a situation where you
might be responsible for providing
lightning safety recommendations or
warning to a group of people.
You should also be aware of the
National
Lightning Safety Institute
,
an organization that is dedicated
to providing accurate lightning safety
information and interested in
lightning safety education.
Lastly I may already have mentioned
the The
30/30 Rule but there
is no harm done repeating it.
Basically
if there is less than 30 seconds
between a lightning flash and the
sound of the thunder, that lightning
discharge is close enough to present a
risk to you. You should be under
cover. You should wait 30
minutes after the last lightning
discharge from a thunderstorm before
concluding that the storm no longer
presents a lightning hazard to you.
Here's a short look at what happens to a
voltage pulse when it arrives at the end of a
transmission line. We look first at the case
of an open circuit (infinite terminating
impedance) at the end of the line.
Pt. a at the top of the figure shows
current flowing from left to right along a signal
wire. This current times the characteristic
impedance of the line produces a voltage pulse
with amplitude Vi shown at Pt. b.
When charge arrives at the open end of the line
the charge must go somewhere. It can't flow
through the infinite impedance at the open end of
the line and it can't just buildup at the end of
the line. The only option is for the charge
to travel back in the direction it came from as
shown at Pt. c. The reflected
current times the characteristic impedance
produces a voltage Vr with the same polarity and
amplitude as the incoming voltage pulse. A
voltage Vi + Vr = 2Vi would be measured across the
open end of the line.
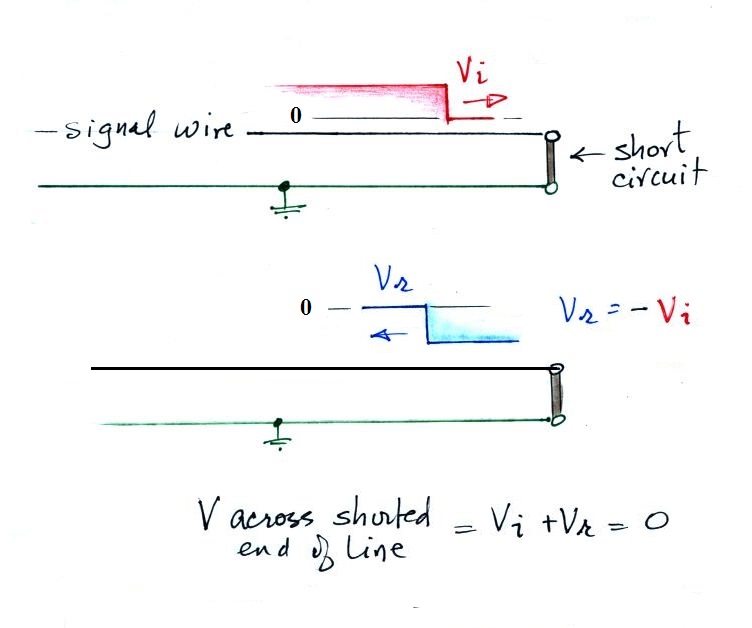
This figure shows the same
voltage pulse, Vi, arriving at the end of a
shorted line. Now the requirement is
that the voltage at the end of the line be
zero. A reflected voltage pulse with the
same amplitude but the opposite polarity is
needed to cancel out the incoming voltage
pulse.
The measured voltage at the end of the line is
zero. The current at the end of the line
has an amplitude that is twice the incoming
current (the reflected current has negative
polarity and is traveling from right to left -
that is equivalent to a positive current
traveling from left to right).
References:
V.A.
Rakov, M.A. Uman, M.I. Fernandez, C.T. Mata,
K.J. Rambo, M.V. Stapleton, R. Sutil,
"Direct Lightning Strikes to the Lightning
Protective System of a Residential Building.
Triggered Lightning Experiments," IEEE
Trans. Power Delivery, 17, 575-585, 2002.
B.A.
DeCarlo, V.A. Rakov, J.E. Jerauld, G.H.
Schnetzer, J. Schoene, M.A. Uman, K.J.
Rambo, V. Kodali, D. Jordan, G. Maxwell, S.
Humeniuk, and M. Morgan, "Distribution of
Currents in the Lightning Protective System
of a Residential Building - Part 1.
Triggered-Lightning Experiments," IEEE
Trans. Power Delivery, 23, 2439-2446, 2008.
R. B. Standler, Protection of Electronic
Circuits from Overvoltages, John Wiley &
Sons, New York, 1989 (republished
in by Dover Publications, New York, 2002)
R.L.
Holle, "Some Aspects of Global Lightning
Impacts," 2015 Amer. Meteorol. Soc. Meeting,
Phoenix, 2015.
J.S.
Jensenius, Jr., "A Detailed Analysis of
Lightning Deaths in the United States from
2006-2013," National Weather Service, NOAA,
2016